How the Ridgecrest earthquake sequence unfolded
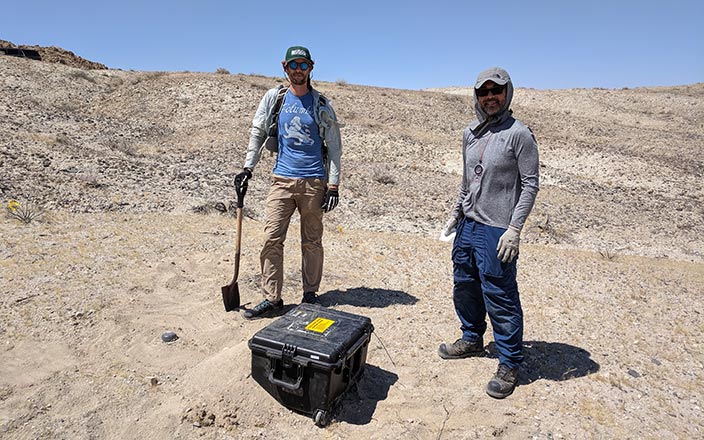
Seismologists at a newly completed seismic station located near the southeastern end of the M7.1 earthquake fault. Photo by Elizabeth Cochran (USGS).
In July 2019 southern California was shaken by the largest quake to occur in the past twenty years, a M7.1 earthquake located near Ridgecrest, California. This was also the first major earthquake since the start of the modern era of the Southern California Seismic Network, which resulted in recording some of the densest and highest quality data of any large earthquake to occur in the United States (Hauksson et al., 2020). Seismologists supplemented these data with hundreds of additional temporary seismic instruments that were installed in the days following the earthquake (Catchings et al., 2020; Cochran et al., 2020). In the year since the sequence began, researchers have been using the seismic data to peer into the Earth to identify which faults moved, and when.
At the time of the Ridgecrest earthquake sequence, it was soon apparent that the sequence had occurred on a complex set of nearly perpendicular faults. On the morning of July 4th, a M6.4 earthquake broke the ground surface mostly along a SW-NE striking fault but produced aftershocks on both SW-NE and NW-SE striking faults (DuRoss et al., 2020). The two faults formed a distinctive ‘L’ shape that was apparent in the early aftershock sequence. Thirty-four hours after the M6.4 earthquake, on the evening of July 5th, a M7.1 earthquake occurred rupturing primarily in a NW-SE direction.
When and where earthquakes occur can be used to determine the order of faults that slipped. Seismologists identified tens of thousands of earthquakes in the first few weeks of the Ridgecrest earthquake sequence. A consistent picture emerges from these studies that shows the sequence activated a complex network of interlocked faults (Ross et al., 2019; Liu et al., 2020; Lomax, 2020; Shelly, 2020). Such tangled fault structures have been occasionally observed in the past, but the large number of precisely located earthquakes available for the Ridgecrest sequence showed an impressive level of complexity. Areas of the rupture that looked simple at first glance were shown to have multiple smaller crisscrossing faults.
The complexity of the faults in the Ridgecrest region likely also controlled, to some extent, the timing of earthquakes in the sequence. The sequence began with a set of small magnitude earthquakes that preceded the M6.4 earthquake by about two hours. These earthquakes were aligned in a NW-SE direction, occurring close to the epicenter of the M6.4 quake. Earthquakes that occurred between the M6.4 and M7.1 events show that the M6.4 earthquake broke across a NW-SE striking fault as well as a shallower SW-NE striking plane. It is this shallower fault that ruptured to the Earth’s surface on July 4th, resulting in minor offsets across Hwy 178. The northwestern tip of the NW-SE portion of the M6.4 rupture came to within just 2 miles southeast of where the M7.1 mainshock began.
In the 34 hours following the M6.4, a set of earthquakes began stepping northwestward from the end of the NW-SE striking slip plane of the M6.4 quake towards the eventual initiation point of the M7.1 (Ross et al., 2019; Lomax, 2020). The largest such event was a M5.4 earthquake that occurred on a cross fault about 16 hours after the M6.4 (Ross et al., 2019; Lomax, 2020). The 34-hour delay between the M6.4 and M7.1 earthquakes suggests that the 2-mile unbroken portion of the fault didn’t permit through-going slip during the M6.4 event. Subsequent earthquakes worked their way up the fault, slowly reduced the size of this gap, and edging closer to where the M7.1 began.
The M7.1 mainshock broke along a NW-SE striking fault, with observable slip at the surface along most of its length (DuRoss et al., 2020) – including breaking across another section of Hwy 178 and causing damage to structures in nearby communities (GEER Team, 2019). Similar to the M6.4 quake, the picture that emerged of the faults that slipped during the mainshock was anything but simple. The SE portion of the rupture broke in two subparallel strands separated by ~3 miles. The fault slip ended less than ~2 miles from the Garlock fault, a large nearly E-W striking fault capable of hosting up to a M7.8 earthquake. To the north, the rupture ended in a mesh of small faults south of the Coso Geothermal field. In total, studies identified at least 10 separate cross-cutting faults oriented both NW-SE and SW-NE (Ross et al., 2019; Shelly, 2020). The complex fault pattern extends across the entire range of depths that earthquakes occurred in the sequence. This is in contrast to observations for many earthquake sequences that showed complex fault patterns at the Earth’s surface tend to merge into a single fault with depth. Intriguingly, one study of the M7.1 earthquake also found evidence that some small earthquakes on closely-spaced, parallel fault strands slipped in opposite directions (Trugman et al., 2020).
The Ridgecrest earthquakes also triggered earthquake swarms in Panamint Valley, on the Garlock fault, and northwest of the Coso geothermal field. These swarms collectively resulted in thousands of smaller earthquakes at considerable distance from the Ridgecrest rupture area. Two of these swarms are still ongoing a year later. A study showed that a majority of triggered earthquakes occurred in regions where the Ridgecrest mainshock added stress that encouraged failure of the local faults (Hardebeck, 2020).
The Ridgecrest earthquake sequence occurred at the southern end of Walker Lane, a region of broad deformation that accommodates about 15-25% of the motion between the North American and Pacific Plates (Wesnousky, 2005). Faults within the Walker Lane as well as the continuation of the zone south into the Eastern California Shear Zone have hosted several large (M7+) earthquakes in historical time, including the 1872 M~7.5 Owens Valley earthquake located 50 miles northwest of the 2019 Ridgecrest sequence.
About the Authors
![]() |
Elizabeth Cochran is an observational seismologist at the U.S. Geological Survey. She serves as Project Chief of the Induced Seismicity project in the Earthquake Science Center. Her research interests include investigating the link between increased earthquake rates and energy production activities. She also conducts research on earthquake early warning, earthquake triggering, rupture processes, and seismic wave propagation, and has led several large seismic deployments following significant earthquakes in the United States. |
![]() |
Zachary Ross is an assistant professor of geophysics at the California Institute of Technology. His current research is focused on developing scalable algorithms for extracting scientifically valuable signals from large seismic datasets using techniques from artificial intelligence and statistics in order to improve understanding of earthquakes and faults. |
Acknowledgements
This research was partially supported by an NSF RAPID Award EAR-1945781 to the Southern California Earthquake Center (SCEC). SCEC is supported under the Cooperative Agreement EAR-1600087 and USGS Cooperative Agreement G17AC00047. We thank our USGS and SCEC colleagues for collaborating in this research.
References
- Catchings, R. D., M.R. Goldman, J.H. Steidl, J.H. Chan, A.A. Allam, C.J. Criley, Z. Ma, D.S. Langermann, G.J. Huddleston, A.T. McEvilly, D.D. Mongovin, E.M. Berg, and Y. Ben-Zion, 2020. Nodal Seismograph Recordings of the 2019 Ridgecrest Earthquake Sequence, Seism. Res. Lett., in review.
- Cochran, E. S., Wolin, E., McNamara, D. E., Yong, A., Wilson, D., Alvarez, M., van der Elst, N., McClain, A., & Steidl, J. (2020). The U.S. Geological Survey’s Rapid Seismic Array Deployment for the 2019 Ridgecrest Earthquake Sequence, Seismol. Res. Lett., doi: 10.1785/0220190296. SCEC Contribution 10117
- DuRoss, C. B., Philibosian, B., Koehler, R. D., Kozaci, O., Ladinsky, T., Madugo, C. M., McPhillips, D., Milliner, C., Morelan, A. E., Olson, B., Patton, J. R., Pickering, A., Hudnut, K. W., Pierce, I., Ponti, D. J., Seitz, G. G., Spangler, E., Swanson, B. J., Thomas, K., Treiman, J. A., Valencia, F., Williams, A. M., Thompson Jobe, J., Hitchcock, C., Gold, R. D., Brooks, B. A., Dawson, T., Scharer, K. M., Kendrick, K. J., Akciz, S. O., Angster, S. J., Bachhuber, J. L., Bacon, S., Bennett, S. E., Blair, L., Bullard, T., Hernandez, J. L., Burgess, W., Chupik, C., DeFrisco, M. J., Delano, J., Dolan, J. F., Frost, E., Graehl, N. A., Haddon, E. K., Hatem, A. E., & Zinke, R. (2020). Surface Displacement Distributions for the July 2019 Ridgecrest, California, Earthquake Ruptures, Bull. Seismol. Soc. Am., doi: 10.1785/0120200058. SCEC Contribution 10110
- GEER Team (2019). Preliminary Report on Engineering and Geological Effects of the July 2019 Ridgecrest Earthquake Sequence, GEER-064, 1–77 p.
- Hardebeck, J. L. (2020). A Stress-Similarity Triggering Model for Aftershocks of the Mw 6.4 and 7.1 Ridgecrest Earthquakes, Bull. Seismol. Soc. Am., doi: 10.1785/0120200015. SCEC Contribution 10118
- Hauksson, E., Yoon, C., Yu, E., Andrews, J. R., Alvarez, M., Bhadha, R., & Thomas, V. (2020). Caltech/USGS Southern California Seismic Network (SCSN) and Southern California Earthquake Data Center (SCEDC): Data Availability for the 2019 Ridgecrest Sequence, Seismol. Res. Lett., doi: 10.1785/0220190290. SCEC Contribution 10121
- Liu, M., Zhang, M., Zhu, W., Ellsworth, W. L., & Li, H. (2020). Rapid Characterization of the July 2019 Ridgecrest, California, Earthquake Sequence From Raw Seismic Data Using Machine-Learning Phase Picker, Geophys. Res. Lett. 47, no. 4, e2019GL086189, doi: 10.1029/2019GL086189. SCEC Contribution 10122
- Lomax, A. (2020). Absolute Location of 2019 Ridgecrest Seismicity Reveals a Shallow Mw 7.1 Hypocenter, Migrating and Pulsing Mw 7.1 Foreshocks, and Duplex Mw 6.4 Ruptures, Bull. Seismol. Soc. Am., doi: 10.1785/0120200006. SCEC Contribution 10123
- Ross, Z. E., Idini, B., Jia, Z., Stephenson, O. L., Zhong, M., Wang, X., Zhan, Z., Simons, M., Fielding, E. J., Yun, S., Hauksson, E., Moore, A. W., Liu, Z., & Jung, J. (2019). Hierarchical interlocked orthogonal faulting in the 2019 Ridgecrest earthquake sequence, Science 366, no. 6463, 346–351, doi: 10.1126/science.aaz0109. SCEC Contribution 10124
- Shelly, D. R. (2020). A High-Resolution Seismic Catalog for the Initial 2019 Ridgecrest Earthquake Sequence: Foreshocks, Aftershocks, and Faulting Complexity, Seismol. Res. Lett., doi: 10.1785/0220190309. SCEC Contribution 10125
- Trugman, D. T., Ross, Z. E., & Johnson, P. A. (2020). Imaging Stress and Faulting Complexity Through Earthquake Waveform Similarity. Geophysical Research Letters, 47(1), doi: 10.1029/2019GL085888. SCEC Contribution 10126
- Wesnousky, S. G. (2005). Active faulting in the Walker Lane, Tectonics 24, no. 3, doi: 10.1029/2004TC001645. SCEC Contribution 10127